Students of IISER Pune next to the strontium-based optical atomic clock’s setup. Photo: IISER Pune.Pune/Bengaluru: Two Pune-based premier research institutes, the Inter-University Centre for Astronomy and Astrophysics (IUCAA) and the Indian Institute of Science Education and Research (IISER), have joined hands to build India’s first two optical atomic clocks.
The institutes will build one clock each, with help from the Government of India. If the project is successful, India will join a small global club of countries with the ability to build these ultra-precise timekeeping devices.
According to the scientists involved, the clocks will only skip one second in more than 13.8 billion years, which is the approximate age of our universe.
“Since the middle of the 20th century till now, there have been tremendous efforts in the field of atomic clocks, making time the most accurately measured physical quantity,” the authors of a paper published in 2014 wrote.
Optical atomic clocks themselves have a few well-known applications. Foremost of course “is accurate timekeeping” – which in turn has multiple applications of its own, according to Subhadeep De, an associate professor and expert in optical physics at IUCAA and one of the members of the project.
For example, GPS satellites use radar signals to determine the position of an object on the ground. However, there is a time lag both due to time taken for the signals to move between the ground and the satellites and because the satellites are in motion relative to the object while they move through Earth’s gravitational field, incurring really tiny but significant time delays arising from the theories of relativity.
The world’s prevailing frequency standard for measuring time is derived from caesium atomic clocks. Here, caesium atoms are imparted energy – by different means in different designs – and forced to jump from one energy level to a slightly higher one, called the atom’s hyperfine ground states. Shortly after, the atom drops back to its previous state by emitting microwave radiation at 9,192,631,770 Hz.
‘Hz’ here is hertz, the SI unit of frequency, defined as ‘per second’. So when a detector measures 9,192,631,770 waves – from crest to trough – of this microwave emission, coming from the caesium atoms, one second will have passed.
According to the Mechatronics Handbook (2002), all timekeeping machines have three parts: an energy source, a resonator and a counter. In a household wall clock, the energy source is a AA or AAA battery; the resonator, in this case the clock’s gears, is the system that moves in a periodic manner; and the counter is the display. The energy and resonator are together called an oscillator.
In atomic clocks, the oscillator is, say, a laser imparting energy to a caesium atom ticking between the two hyperfine ground states. The radiation the atom releases is the resonator. The detector is the counter.
The clocks being built by IUCAA and IISER have the same underlying principle but use more advanced technologies. Indeed, optical atomic clocks are considered to be the next step in the evolution of atomic clocks and are likely to replace caesium atomic clocks as the world’s time standard in future. A glimpse of the underlying engineering shows us why.
First, confining the atoms or ions is very difficult. To keep the clock precise, its operators need to ensure the atoms don’t combine to form molecules, bump into each other and/or don’t react with the container’s walls. So instead of confining them in material containers, the IUCAA and IISER teams are using optical and electromagnetic ‘traps’.
Specifically, “neutral atoms are confined in an optically created … ‘storage basket’ known as an optical lattice, which is created by interfering two counter-propagating laser beams,” Umakant Rapol, an associate professor at IISER, said. The ions are confined by oscillating electric fields.
Second, once the particles have been confined, they will be laser-cooled to nearly absolute zero (the coldest temperature possible, 0 K or -273.15º C). In their simplest form, laser-cooling techniques force atoms to lose their kinetic energy and come very nearly to a still. Since the temperature of a macroscopic body is nothing but the collective kinetic energy of its atoms, a container of nearly-still atoms is bound to feel very cold. And once more of the atoms’ kinetic energy has been removed, their quantum physical effects become more noticeable, allowing the clock to be more precise.
The choice of atoms to use in the clock is dictated by whether they can be cooled to a few microkelvin above absolute zero using laser-cooling, and if their switching between the two energy states “is immune to stray magnetic fields, electric fields, the temperature of the background, etc.,” Rapol said.
Ytterbium and strontium atoms check both these boxes. IUCAA will be building a ytterbium-ion clock. In this clock, a single ytterbium ion will be used to produce the resonating radiation. Using multiple ions “gives rise to an effect called a Coulomb shift,” which interferes with the clock design. IISER will be building a strontium-atom clock.
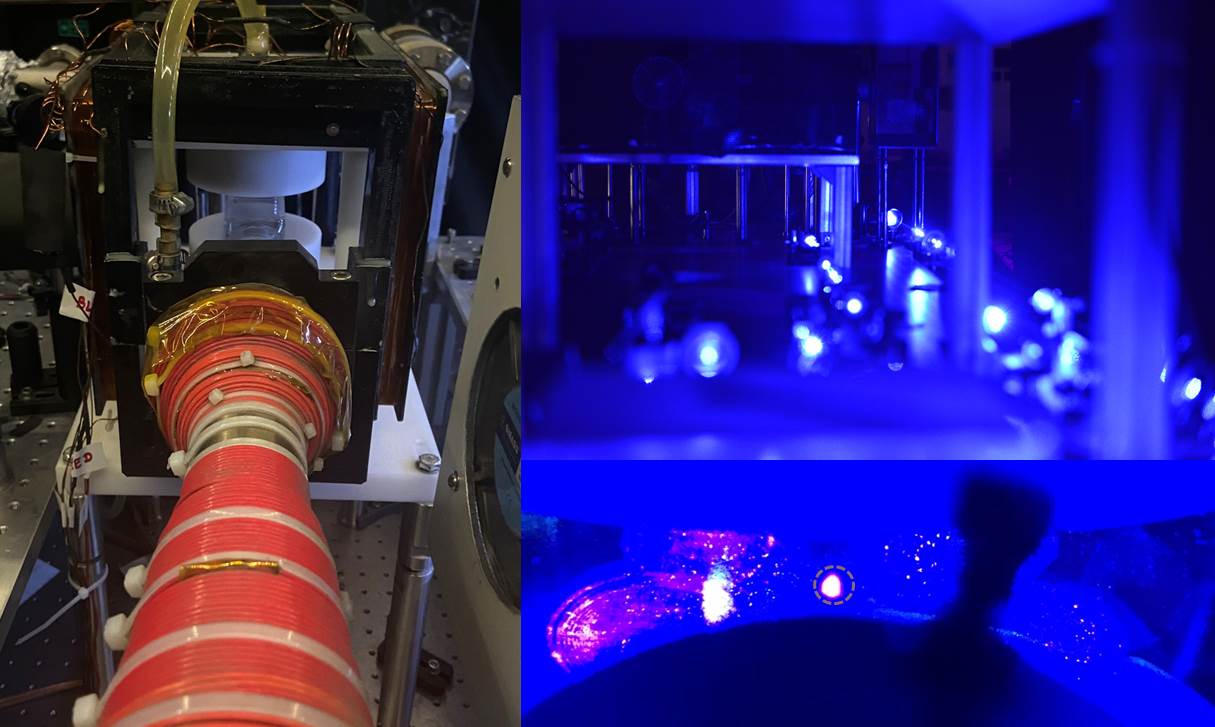
When a caesium atom swings between the two hyperfine ground states, it emits a specific amount of energy as microwave radiation. When the ytterbium and strontium atoms swing between two of their energy states, they emit energy as optical radiation. Both these elements have highly stable optical emissions at wavelengths of 467 nm and 698.4 nm – corresponding to 642,121,496,772,645 Hz and 429,228,066,418,009 Hz for ytterbium-ion and strontium atom, respectively.
These high frequencies – two orders of magnitude higher than the microwave radiation in caesium clocks – is the source of the clock’s ability to miss less than one second in 13.8 billion years.
(The makers of an optical strontium clock reported in 2014 that their device wouldn’t miss one second in 15 billion years!)
Also read: Experimenting with Cold, Magnetic Materials in Indore
However, taking advantage of this stable emission means accurately detecting the high-frequency optical radiation. That is, if researchers need to build optical atomic clocks, they also need to be able to build and operate state-of-the-art frequency measurement systems. These devices – in the form of frequency combs – constitute the third feature of the IUCAA and IISER clocks.
A frequency comb is an advanced laser whose output radiation lies in multiple, evenly-spaced frequencies. This output can be used to convert high-frequency optical signals into more easily countable lower-frequency microwave signals – like in the diagram shown below (source).
The principal challenge before India is to build all these devices from scratch. Rapol said the teams plan to “develop most of the required technologies” in Pune. They require expertise in the fields of optics, instrumentation, electronics, ultra-high vacuums, and mechanical and software engineering, among others.
“National collaborations – such as [us] working together with our next-door neighbour IISER – will be beneficial,” De said. Rapol mirrored this opinion: “We are going to share expertise with IUCAA and are already working [together] to create an ion trap.”
Rapol also said one clock is half-ready: “We have laser-cooled the strontium atoms and are ready to load these atoms into one-dimensional chains, to increase the signal-to-noise ratio, and will have the optical clock soon,” he said. They are also waiting to fit in the frequency comb.
He estimated that once the funds and equipment have been procured, it should take two years or less to build the clock at IISER. The IUCAA clock is expected to be ready in four or five years.
Once both clocks are operational, they will be linked together.
Grander applications
There are multiple open problems in physics at the moment. Four of the more prominent ones include the search for ‘new physics’, the reconciliation of quantum mechanics and relativity, an explanation for what happened to the universe’s antimatter, and the nature of dark matter.
De noted that various experiments designed to help answer these questions – and others besides – require researchers to be able to measure time in different contexts with increasingly higher precision and accuracy.
Rapol also expressed excitement about measuring changes in the values of fundamental constants. Constants are called so because their values don’t change – but the values of some constants could be changing too slightly for existing clocks to notice.
For example, the fine-structure constant is a number that determines the strength with which a charged particle, like an electron or a ytterbium ion, couples with an electromagnetic field. If this number increases or decreases with time, there could be implications for the whole universe – everywhere charged particles interact with each other.
According to De, the ytterbium ion is more sensitive to the fine structure constant than strontium atoms. So if the constant’s value changes with time, the ytterbium clock’s transition frequency “will vary at a much faster rate” relative to that of the strontium clock. “This [difference] will eventually allow us to measure time variation of the fundamental constant, if there is any at all.”
For a different example, physicists who study particles called neutrinos sometimes need to beam these particles from a source to a detector hundreds of kilometres away, through the atmosphere (these particles are entirely harmless). In 2011, physicists in Italy found that some neutrinos that had been beamed from a facility near Geneva and detected at their instrument, called OPERA, had travelled faster than light. The claim became a major source of controversy because faster-than-light travel violates the special theory of relativity.
The problem was found a few months later: the OPERA master clock had glitched, and measured the neutrinos’ time of arrival wrong by just 75 nanoseconds.
Other applications of atomic clocks include GPS systems, gravity-aided navigation, astronomy and geology.